Shaowei Guo,†‡# Lior Debbi,†# Barak Zohar,† Roee Samuel,† Roni S. Arzi,₴ Adina I. Fried,† Tahel Carmon,† Dudi Shevach,† Idan Redenski,† Inbar Schlachet,₴ Alejandro Sosnik,₴ Shulamit Levenberg†*
† Faculty of Biomedical Engineering, Technion-Israel Institute of Technology, Haifa, Israel
‡ The First Affiliated Hospital, Shantou University Medical College, Shantou, China
₴ Laboratory of Pharmaceutical Nanomaterials Science, Department of Material Science and Engineering, Technion-Israel Institute of Technology, Haifa, Israel
Shaowei Guo’s email: 07swguo1@stu.edu.cn; mobile: 13923961086
*Corresponding author: Shulamit Levenberg Email: shulamit@bm.technion.ac.il
Background: Extracellular vesicles (EVs) have emerged as a promising strategy to promote tissue regeneration. However, overcoming the low EV production yield remains a big challenge in translating EV-based therapies to the clinical practice. Current EV production relies heavily on 2D cell culture, which is not only less physiologically relevant to cells, but also requires substantial medium and space. Therefore, we aimed to boost EVs secretion by a novel method combining tissue engineering and biomechanics.
Methods: In this study, we fabricated and characterized 3D scaffolds, and engineered 3D tissues by seeding on the scaffolds human stem cells from dental pulp or adipose tissues, or skeletal muscle cells. Then, we applied two-forms of mechanical stimuli, including flow and stretching, of different mechanical strengths to the 3D tissues grown in bioreactors. EVs secretions from those 3D tissues were quantified by using Nanosight equipment, and their cargos were characterized by proteomics. Next, we investigated the mechanisms behind the change in EVs secretion by staining with Yes-associated Protein (YAP), a marker of mechanosensitivity. Finally, we compared the neurotrophic effect on primary neurons between mechanically-stimulated EVs or their 2D counterparts.
Results: Under computational flow dynamics analysis, we quantified the shear stress within the scaffolds that the cells sensed for producing EVs. The EVs secretions were significantly increased, up to 150-fold higher, with optimized mechanical stimulations, and their altered cargos were highly related to the biogenesis of EVs secretion. Further mechanistic investigation revealed that this process was mediated by YAP mechanosensitivity. Mechanically-stimulated EVs displayed superior capability in inducing axonal sprouting than the 2D counterparts.
Conclusions: To conclude, we demonstrated the effect of mechanical stimulations on boosting EVs production from 3D engineered tissues mechanically stimulated under bioreactors. Our results demonstrate the promise of this strategy to boost EV production and optimize their functional performance towards clinical translation.
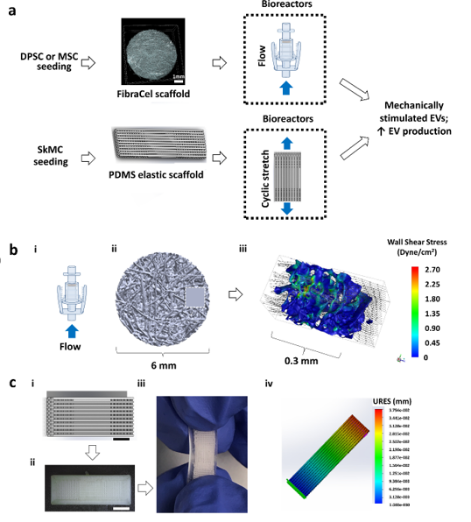
Figure 1. Engineered tissues loading systems for extracellular vesicles production. (a) Process diagram of the production of mechanically stimulated EVs from dental pulp stem cells (DPSCs), mesenchymal stromal cells (MSCs), or skeletal muscle cells (SkMCs)-seeded engineered tissues exposed to flow stimulation or cyclic stretching within bioreactors. The blue arrowheads denote the directions of flow or cyclic stretching within the bioreactors. (b) Schematic of the application of flow shear stress on 3D engineered tissues for EVs production. (i) Flow bioreactor (ii) Micro-CT scaffold for shear induced EVs production (iii) Computational Fluid Dynamics (CFD) simulation for wall shear stress applied on scaffold representative segment. (c) Schematic of the application of cyclic tension forces on 3D engineered muscle tissues for EVs production. Scale bar = 3 mm. (i) Stretchable scaffold design with defined fiber orientation (ii) BVOH printed mold for scaffold fabrication (iii) PDMS elastic scaffold with defined fiber orientation (iv) Finite Element Analysis (FEA) analysis for applied displacement map during loading.
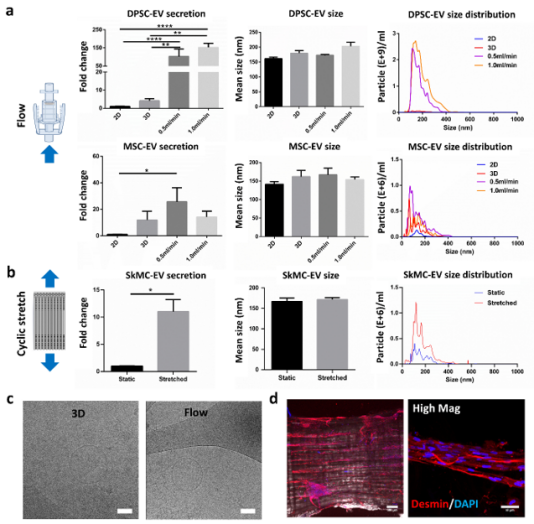
Figure 2. The influence of mechanical loading on EV production. (a) EV production (left), size (middle) or size distribution of DPSCs under 2D (n=7), 3D static (n=11), 0.5 ml/min (n=3), or 1.0 ml/min (n=8) conditions, and of MSCs under 2D (n=3), 3D static (n=7), 0.5 ml/min (n=5), or 1.0 ml/min (n=4) conditions. (b) EV production, size and size distribution of stretched or unstretched SkMC scaffolds. (c) Cryo-EM imaging of EVs from 3D static or flow group displaying typical cup-shape structure. (d) Immunofluorescence imaging of myotube formation on engineered skeletal muscle tissues. Higher magnification image shown on the right. Desmin (red) and DAPI (blue). Scale bar = 100 µm and 10 µm.
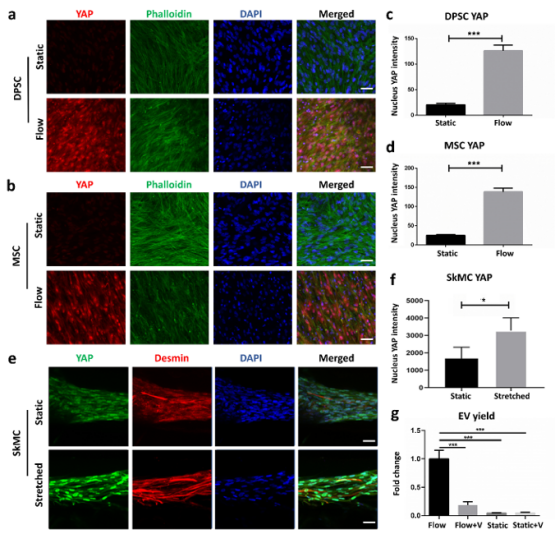
Figure 3. Mechanical loading application for EV production mediated by YAP mechanotransducer. (a, b) Immunofluorescence imaging of DPSC or MSC embedded scaffolds under static or flow stimulation. YAP (red), Phalloidin (green), DAPI (blue). Scale bar = 50 µm. (c, d) Quantification of nucleus YAP intensity of DPSC or MSC-embedded scaffolds under static or flow stimulation. N=3/group. Two-tailed Student’s t-test. *p<0.05, ***p<0.001. (e, f). Immunofluorescence imaging and quantification of nucleus YAP intensity of SkMC-embedded scaffolds under static or stretched stimulation. YAP (green), Desmin (red), DAPI (blue). Scale bar = 50 µm. (g) EV yield of DPSC embedded in 3D scaffold under flow stimulation, flow with YAP inhibitor- Verteporfin (Flow+V), static control, and static with Verteporfin (Static+V). One-way ANOVA with post-hoc Tukey’s multiple comparisons. ***p<0.001.